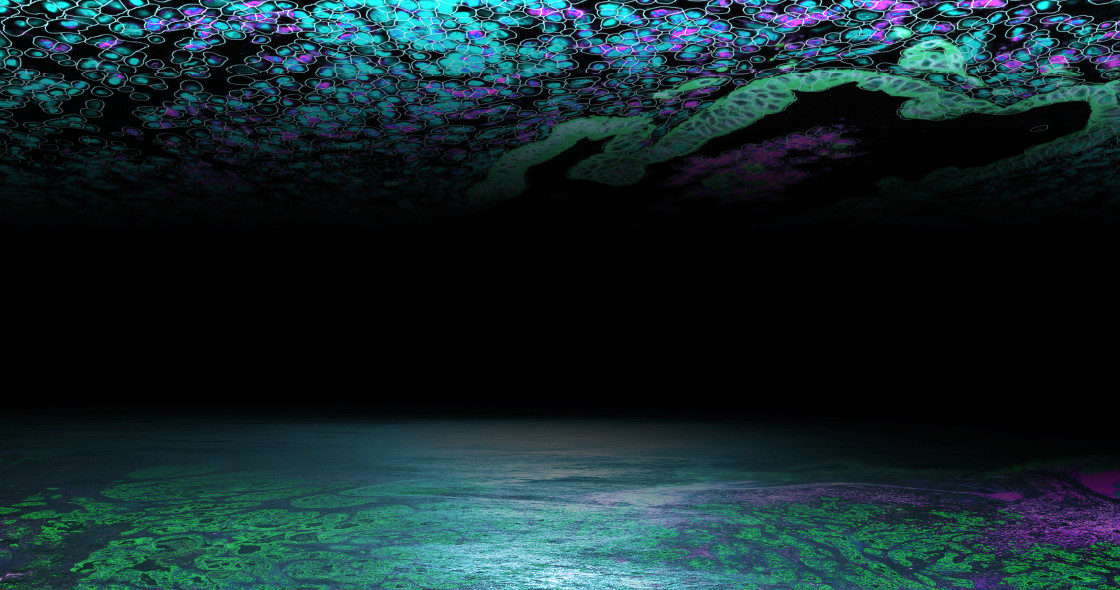
Understanding Spatial Sequencing in the Context of Spatial Biology
What is spatial sequencing?
Spatial sequencing is viewing a specific area or a region within a tissue, then sequencing all transcripts found in that region. Spatial sequencing allows the user to map variation in gene expression across a tissue or region of interest.
Spatial organization and spatial relationships are an essential part of how organisms function. Lack thereof or loss of spatial orientation can lead to a loss of biological function. Spatial organization is important at a variety of levels. It could refer to the locations of organs relative to one another. For instance, it doesn’t make sense to have a pancreas, which is involved in digestion, located near the brain in a human. It can also refer to subcellular architecture in tissues, the spatial relationships of different cells to one another, or the spatial organization of macromolecules within a cell.
Spatial sequencing leverages two common molecular biology techniques to ascertain transcriptional dynamics in a biologically relevant context. The first of these techniques is microscopy. Using immunofluorescence microscopy and either labeled antibodies or RNA probes, the user can select a region of interest. The user can then shine a UV light on the sample and cause tags to be released by the antibodies or RNA probes. These tags can then be processed through the second technique, next-generation sequencing, to determine the transcripts present. This combinatorial effort reveals an additional level of granularity, ultimately providing new insights into the biological context of transcriptional dynamics. Spatial sequencing can be leveraged at the tissue, single cell, or subcellular levels. Depending on context, spatial sequencing may also be called spatial profiling, spatial biology, or as Nature Methods referred to it when choosing it as method of the year in 2020, spatially resolved transcriptomics.
Regardless of the term chosen, biologists have utilized spatial sequencing in a variety of contexts. For example, within cancer biology research, researchers have examined several cancer types, including breast cancer and colorectal cancer. For example, one group compared gene expression between low- and high-grade tumors. Others are using spatial sequencing to generate a spatial organ atlas. Like an atlas of maps, the spatial organ atlas would serve as reference material for those trying to orient themselves in an unfamiliar plane. In this case, researchers would be able to understand how transcriptional dynamics change in a healthy organ. For example, how gene expression may vary between different functional regions of an organ. With this ‘map’ in hand, researchers can better understand how transcriptional dynamics change in, for example, disease states. A better understanding of disease progression could ultimately lead to better treatment options.
What are the advantages of single-cell sequencing?
The advantages of single-cell sequencing are that it allows the users to look at specific, unique, or rare cell types within their specific biological context. Previous bulk sequencing experiments that generalized across tissues resulted in the loss of important signals. With single-cell sequencing, individual cells can be chosen and studied based on the research question.
Single-cell sequencing is a subtype of RNA sequencing or RNA-Seq. RNA-Seq is a method that leverages next-generation sequencing to understand global transcriptional dynamics in a cell. Since RNA-Seq uses next-generation sequencing technology, the user is not required to know all gene targets at the onset of the experiment. Therefore, RNA-seq can be used to identify novel transcripts.
Depending on the library preparation, the user may also choose to look only at coding RNAs or both coding and non-coding RNAs. Researchers can also garner information such as splice variants or other post-transcriptional modifications.
Traditional batch sequencing in RNA-seq is useful for understanding biological processes across tissues. For example, understanding how transcriptional dynamics in a particular cell type respond after treatment with a drug. Although useful and certainly important depending on the context and specific research question, batch sequencing also generalizes all transcripts together. This can result in the loss of biologically important information as it will be lost in favor of the more abundant transcripts.
Therefore, a major advantage of single-cell sequencing is that researchers can focus on a specific cell type or intentionally select rare or unique cells to study. Researchers can also individually characterize all cells in a tissue sample to understand the overall composition and heterogeneity of the system. Researchers can also track how heterogeneity changes over time, such as when cells become cancerous. Notably, researchers can ask these questions with relatively small populations of cells, as low as in the hundreds of cells. Therefore, single-cell sequencing has the advantage over bulk sequencing in that it provides a finer resolution on the identities of cells within a specific tissue. The information obtained is more fine-grained and provides a different viewpoint on the biology of tissues.
What is the difference between single-cell RNA-Seq and RNA-Seq?
The difference between single-cell RNA-Seq and RNA-Seq is workflow. In standard RNA-Seq, all cells from a tissue are maintained in one sample, and these transcripts are sequenced. This gives the user an overview of transcriptional dynamics in a tissue. In single-cell RNA-Seq, cells are sorted first, then specific cells of interest are sequenced.
RNA Sequencing or RNA-Seq is a method used for studying the whole transcriptome. Since it relies on next-generation sequencing, users can obtain information about known and unknown sequences. Researchers have utilized RNA-Seq in a variety of contexts. For example, researchers may examine transcriptional dynamics before and after drug treatments. One could also compare transcriptional dynamics between different cell types, tissue contexts, or in healthy versus diseased states. RNA-Seq also affords the investigator the ability to examine splice variants, post-transcriptional modifications, and SNPs. RNA-Seq is superior to microarrays since sequences do not previously need to be known, and assessment of gene expression level is superior.
RNA-Seq works by first preparing the library. Nucleic acids are purified from a tissue sample, then DNA is removed. From here, the RNA library is processed according to the desired output. For example, one may choose to keep only coding RNAs by selecting RNA species with a poly-A tail. Once RNA selection is complete (if desired), the RNAs present are used to generate a cDNA library. These cDNAs are indexed with a barcoding system. Single-cell RNA-seq follows the same procedures, except that rather than starting with a tissue containing many kinds of cells, the user starts with a single cell. Traditional bulk RNA-Seq may be used to answer research questions such as how to analyze transcriptional dynamics change in a tissue before and after treatment. In single-cell RNA-Seq, the user can assess biological differences between distinct cell types. One could also assess how cells change over time during a process, such as during differentiation.
How does spatial sequencing work?
Spatial sequencing works by combining immunofluorescence with next-generation sequencing. Samples are stained with fluorescently labeled antibodies or probes. Using a spatial biology setup, the user selects a region of interest, then uses ultraviolet light to release molecular tags. These tags are then processed using next-generation sequencing.
Spatial sequencing relies on two common molecular biology techniques, immunofluorescence microscopy and next-generation sequencing. The combination of the two techniques provides unparalleled insights into spatially resolved transcriptomics.
Using either formalin-fixed paraffin-embedded (FFPE) or fresh-frozen samples, the user can add either fluorescently labeled antibodies or RNA probes, depending on the research question of interest. Once staining is complete, using a spatial biology microscopy setup, the user can scan through the samples. For example, the user may be interested in a particular functional region of a tissue, structural feature, or interface between cell types. Once a region of interest is identified, the user shines a UV light onto this region. This UV light causes tags linked to the antibody or probes by a photocleavable linker to release. The user can then collect these tags and process them using next-generation sequencing.
With next-generation sequencing, scientists can analyze tens of thousands of targets. Scientists do not need to know the identity of targets at the beginning of an experiment, allowing the identification of novel targets. Depending on the technology used, cell type of interest, and if the research requires protein or RNA, the user requires between 5 and 200 cells total. Using this technology, scientists have uncovered many new insights into molecular biology. In the context of cancer biology research, scientists have examined transcriptional dynamics in breast cancer, the variation in gene expression between different regions of a tumor and lymph nodes, and the transcriptional dynamics between tumor and immune cells. Other groups have set out to create a spatial organ atlas. The goal of the atlas is to create a reference describing gene expression in a three-dimensional, non-diseased organ. This will provide a benchmark of comparison when trying to understand disease processes.
For Research Use Only. Not for use in diagnostic procedures.